Synthetic biology is a chance to engineer the biological world.
Words: Kim Thomas / Photography: Joe McGorty
Imagine you could program a living cell in the same way you currently program a computer. Cells could be made to convert carbohydrates to fuel – or to detect disease-causing bacteria. Plants could be programmed to create new, life-saving drugs. Welcome to synthetic biology – less than 20 years old and already making an impact. Its logic is irresistible: take engineering principles – breaking material down into modules and combining them in different ways – and then apply them to biology.
It’s a bit like turning living cells into little manufacturing factories: and the diversity of things these factories can produce and the precision you can apply is endlessly exciting. “Humans have been using biology for more than 5,000 years,” says Professor Paul Freemont, Co-Director of the EPSRC Centre for Synthetic Biology and Innovation (CSynBI), “but synthetic biology now offers the opportunity to rationally engineer biology for the first time.”
In practical terms, scientists take sequences of DNA and put them together in different combinations within a cell, thereby programming cells to behave in new ways. “The whole field is about how you write DNA within the context of a particular cell type, to make the cell produce something you want according to human design,” says Professor Richard Kitney, fellow Co-Director of the CSynBI.
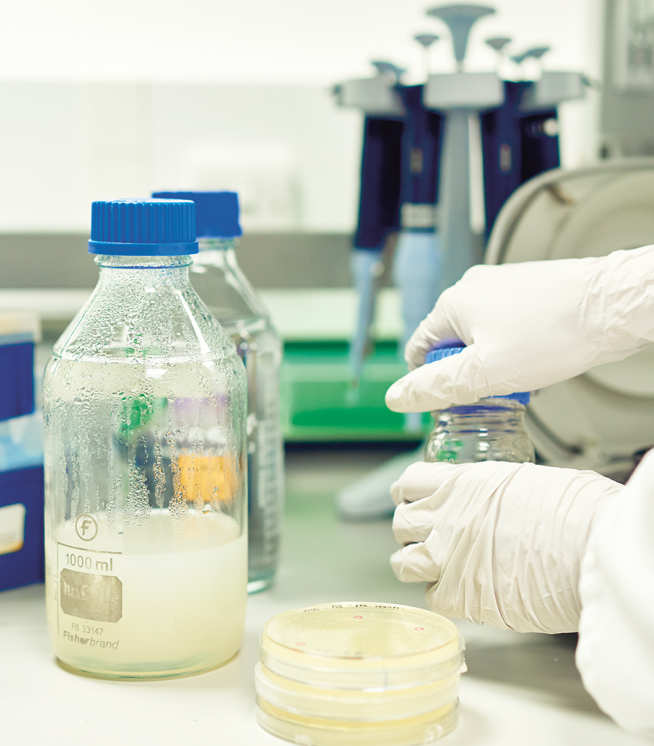
As well as anti-malarial drugs and modified yeast-producing biofuels, Kitney believes one of the most promising applications is vaccine production. It normally takes nine months to produce a vaccine, but with synthetic biology they can be produced in only one month in plant cells: “You take the plant cell, modify its DNA and then get the plant to produce the vaccine. Harvest the plant and grind it up – then the liquid that comes out of that grinding process is the actual vaccine.”
Speeding up process is also at the heart of PhD student Loren Cameron’s work. She is developing a revolutionary new biosensor – a piece of biological material that converts a biological response into a detectable signal – to help sufferers of cystic fibrosis, a chronic disease caused by a genetic mutation. Sufferers are highly susceptible to respiratory infections such as Pseudomonas aeruginosa and, currently, it can take 24 hours or more for patient sputum sample cultures to detect the presence of the Pseudomonas bacterium and other pathogens.
What’s special about Cameron’s biosensor is that it uses cell-free synthetic biology to shortcut the process and detect P. aeruginosa more quickly and more cheaply. “You take all the cellular machinery out of the context of a living cell, so it’s a non-living system,” she explains. Living cells are highly complex, making them difficult to engineer.
And the great advantage of a cell-free system is that it can be freeze-dried and reactivated: “There’s no issue of molecules crossing a cell membrane because everything is open and exposed and ready to sense whatever is in your sample.”
Early testing with clinical samples has shown that the biosensor works, and can quantify P. aeruginosa-specific molecules with reasonable accuracy compared to analytical methods. The research demonstrates the potential of cell-free biosensors in the diagnosis of bacterial infections, particularly, says Freemont, in low- and middle-income countries that cannot afford sophisticated diagnostic equipment. Longer term, he says, the technology could be used to build “low-cost diagnostic sensing devices that could be used to detect viral infections, bacterial infections and environmental pollutants”.
Whether it’s creating vaccines or detecting Pseudomonas, the holy grail for synthetic biologists lies in a greater understanding of genomes – the complete set of genetic material in a living cell. We can already ‘read’ a genome – that is, break it into its component parts, consisting of thousands of tiny pieces of DNA. But Imperial is part of a project that goes one step further: it is helping to write a genome.
Anyone who appreciates bread or beer knows how important yeast is, particularly its ability to convert carbohydrates to carbon dioxide and alcohols through fermentation. Now, an international collaboration, known as the Synthetic Yeast Genome Project, aims to build a synthetic version of the entire yeast genome, an accomplishment that is centrally important in modern cell biology research.
Each of yeast’s 16 chromosomes has been allocated to teams of scientists across the globe. The Imperial team, led by Dr Tom Ellis, has been working on chromosome 11, a middle-sized chromosome with 700,000 bases of DNA. Their work consists of editing synthetic DNA sequences into chunks then introducing them into yeast cells, where existing cellular machinery finishes building the chromosome. Eventually – possibly as soon as the end of 2018 – all 16 chromosomes will be brought together to make an entire genome.
For the very first time, it is possible to harness the biological sphere according to human design"
If the project was just about replicating the existing yeast genome, then its usefulness would be limited. But the scientists are making slight changes as they go, removing some unnecessary genetic material and adding features that might improve on the original, says Ellis. “We’ve done a lot of learning by breaking down the natural systems – let’s see if we can learn even more by building up a new version of the natural system based on the knowledge we have.”
As a result of the changes to the genetic code, the finished yeast chromosome will have what Ellis calls “special powers” – new strains which make the bread-making or beer-making process more efficient. But the project is also improving scientists’ understanding of how to program yeast to produce, for example, medicines or fuels. The process of building the yeast genome will also make it easier to build other genomes, such as those of plants or animals. Finally, because yeast shares a quarter of its genes with humans, understanding how yeast works will also help us understand the human genome better.
Owning life
Synthetic biology is not without challenges. It’s all very well using yeast or bacteria cells to make a medicine in a lab, but scaling it up to a factory process is much harder. And there are ethical questions about the potential harm of rewriting genetic code, as well as concerns about intellectual property and whether a living organism can be ‘owned’.
The government’s Synthetic Biology Leadership Council (SBLC), which decides priorities and recommendations for synthetic biology research in the UK, is looking specifically at the ethical and regulatory issues. Companies that provide DNA sequences on request to researchers have monitoring programmes to ensure that no-one submits a design that could inadvertently cause harm. But the complexity of what’s involved should not be underestimated.
However, it is wrong to imagine that scientists are able simply to put DNA sequences together to build a new biological system. The term BioBricks (standardised pieces of DNA sequences), gives the misleading impression, says Freemont, that they are “like bricks of Lego you can stick together”. A living organism is “a very constrained volume of chemical reactions” which creates context dependencies: “You can’t just say: ‘If I put these parts together it’s going to work’.”

A growing, open access database now exists of those DNA sequences, known as the Registry of Standard Biological Parts, which contains information about every BioBrick (or biopart, to use the term preferred by Imperial scientists). “When a particular biopart is defined, it goes through a detailed experimental procedure to describe exactly how it operates under various conditions,” says Kitney. Once that has been determined, all the details are put into the Registry.
Truly understanding the interaction between different biological components is far from being a short-term project. The team led by Dr Guy-Bart Stan, Head of the Control Engineering Synthetic Biology group, is developing mathematical models that more effectively predict what happens when new DNA is inserted into a cell. “That will allow us to increase the reliability and the predictability of what will happen before we perform the experiment,” he says.
“When engineers design a new aeroplane on a computer, the information about how the different elements interact and behave is so reliable that the computer modelling can be used to build a single prototype that is instantly successful,” says Stan. “So they don’t build 10,000 planes and then select the one that flies correctly. It’s totally cost-ineffective to do that. The long-term vision is to reach a stage where we can design or engineer living cells in a way akin to what we do in manmade systems when we build a plane.”
It’s still a young field, and that vision is some years off. But the potential is undoubtedly vast and, as Kitney says, it represents a huge leap forward in what is scientifically possible: “For the very first time in the history of the world, it’s possible to begin to harness the biological sphere to operate according to human design.”